Keywords |
Juglans regia L., Walnut milk, Anticancer activity, Apoptosis, Breast cancer, Prostate cancer |
Introduction |
Human health studies have revealed that there is a clear
significant correlation between the regular consumption of
natural products and a reduced incidence of several diseases,
such as physiological disorders and lung, oral-pharynx, colon,
pancreatic and endometrial cancers [1]. These anticarcinogen,
radioprotective and chemopreventive characteristics have been
attributed to the content of natural flavonoids (especially
quercetin, mrycetin, naringenin, and apigenine), lectines,
polyphenols, anthocyanin, and vitamins (ascorbic acid and
tocopherols) [2-5]. Antioxidant and preventive roles of
phenolic compounds was reported from in vivo and in vitro
studies, and they are thought to be major bioactive molecules
with human health benefits [6-8]. |
Walnuts, Juglans regia L., are commonly found in temperate
areas of the Palearctic, Nearctic and Oriental zones and are
commercially cultivated in several countries. In all cultivated
areas, the shrubs, seeds, shells, bark, green husk and leaves of
walnuts are used in complementary medicine and the
pharmaceutical and cosmetic industries [1]. Several parts of
walnuts are good sources of phenolic compounds [8-10]. Several phenolic molecules reduce the molecular damage
associated with degenerative diseases by preventing cellular
oxidative stress and inhibition of macromolecular oxidation
[5,9,11,12]. Recent studies demonstrated the antioxidant,
antiradical, antimicrobial and antiproliferative activity of
phenolic products using different in vitro and in vivo estimation
models [1,13-15]. |
Walnut milk (U can Adam Company, Turkey) is a novel walnut
drink that is obtained by mixing fresh walnut sap with certain
amounts of an aqueous extract of male flowers and internal
membranes of fruit. This drink is patented by the Turkish
Patent Office (Patent No.: TR 2010/06465- B) and is legal for
human consumption as a dietary supplement. Some studies
reported the potential antioxidant, antimicrobial, antiradical
and antiproliferative effect of walnut extracts and fruits
[9,15,16]., leaves [1], and liqueurs produced from green
fruits[17] and the green husk [1,9], but information about the
male flower and internal membrane of fruits is almost nonexistent.
In addition, the combined anti-carcinogenic effect of
extracts of several parts of walnuts has not been studied. |
In this paper, we determined the polyphenol content and the
amounts of quercetin and juglone and evaluated the cytotoxic
and anticancer activity of walnut milk in DU145, MCF7 and
TG/HA-VSMC cell lines. We also demonstrated for the first
time to our knowledge the apoptotic affect and the role of the
intrinsic apoptosis signalling pathway of walnut extracts on
healthy and cancer cell lines via image-based cytometer and
target gene expression profiles. The aim of this study was to
investigate the genetic mechanisms of the anticarcinogenic
properties of a special walnut mixture (walnut milk) as a
potential anticancer treatment. |
Materials and Methods |
Chemicals |
Three cell lines, human prostate carcinoma DU 145 (ATCC®
HTB-81™), human metastatic breast adenocarcinoma MCF7
(ATCC® HTB-22™), and human normal aorta smooth muscle
TG/HA-VSMC (ATCC® CRL-1999™) were purchased from
the American Type Culture Collection (ATCC, Rockville,
Maryland, USA). The cell culture materials HAMS F 12,
Dulbecco’s modified Eagle’s medium (DMEM), L-glutamine,
foetal bovine serum (FBS), and penicillin-streptomycin were
supplied by MULTİCELL (Vısent Bioproducts, Canada). PBSEDTA,
dimethyl sulfoxide (DMSO), trypsin, yellow 3-(4,5-
dimethylthiazol-2-yl)-2,5-diphenyltetrazolium bromide (MTT)
and analytic standards for the phenolic compounds, quercetin
and juglone were purchased from Sigma-Aldrich Chemical Co.
(St. Louis, MO, USA). The PureLink® RNA Mini Kit, High
Capacity cDNA Reverse Transcription Kit, SYBR® Select
Master Mix and Tali® Apoptosis Kit - Annexin V Alexa
Fluor® 488 and propidium iodide were supplied from Life
Technologies (USA). Methanol, acetonitrile and ultrapure
water (LiChrosolv® Reag) were purchased from Merck-
Millipore (Darmstadt, Germany). |
Walnut milk |
The walnut milk and the extraction procedures were supplied
by the manufacturer (Ucan Adam Inc. Turkey). This procedure
is also found in the patent document (Patent No. TR
2010/06465- B, Turkish Patent Institute) and is given below.
Walnut sap was collected from approximately 24 cm in the
trunk of a walnut tree and stored in a stainless steel tank at
<4°C until it was used. Walnut male flowers and the inner
membrane of walnut fruits were collected in the Denizli
province of Turkey. After collection, these materials was washed with distilled water and mechanically cut in to very
small parts and dried at room temperature in the dark. Shade
dried plant materials were powdered with a tungsten carbide
bit to approximately 60-mesh size and used for water
extraction. Walnut milk was obtained from fresh walnut sap
with certain amounts of an aqueous extract of male flower and
the internal membrane of walnut fruits. This walnut extract was
subsequently bottled and stored at <4°C. |
Determination of polyphenols, plant hormones,
quercetin and juglone |
During plant chemical analysis, standards for the phenolic
compounds and the plant hormones quercetin and juglone were
individually loaded onto the micro liquid chromatography
(Exigent Micro LC 200, Abi-Sciex, USA)-Electron Spray
Ionisation-Triple Quadropol-Time of Flight Spectrometer
(Micro LC-ESI- Q-TOF, Abi-Sciex, USA) system to determine
fragments and analysis conditions. Afterwards, mixtures of the
15 analytic standards were prepared with concentrations
ranging from 0.100 to 200 μg/kg to set up eight points for
calibration. All data were opened with Peak View (ABI-Sciex,
USA) software, and mass and fragment results were checked
with Master View (ABI-Sciex, USA) options. Mass and
specific fragments were exported to MultiQuant software
(ABI-Sciex, USA) to generate calibration curves for each type
of compound. Samples (2 μl) were analysed by Micro LC-ESIQ-
TOF using an Eksigent MicroLC 200 Plus system coupled
with an Applied Biosystems 4600 Triple Quadropol-TOF.
Chromatographic separation was carried out on a Eksigent 2.7
μ*3 cm C18 halo column at 30°C. The solvent gradient was as
follows: 100 % A (99.8% UPW: 0.2% formic acid) to 100% B
(99.8% acetonitrile: 0.2% formic acid) over 10 min. The
gradient profile for plant chemicals was applied as follows: (t
(min)/A%): (0/90), (1/90), (2/10), (6/10), (7/50), (9/90),
(10/90). Phenolic compounds, hormones, quercetin and juglone
analyses were performed using a DuoSpray source and
Electrospray (ESI) probe. An IDA method was used containing
a TOF-MS survey of 70 ms and up to 20 dependent TOFMS/
MS scans of 25 ms accumulation time. The mass range
was set to 100-960 da for MS and the product ion mass range
was set to 30-960 da for MSn. The curtain gas was set at 20
a.u.; the source temperature was 400°C; and the ion source
gases 1 and 2 were both 30 a.u. The declustering potential was
set at -100 V. The source voltage was -4500 V. A Collision
Energy (CE) of -20 V and Collision Energy Spread (CES) of
-15 V were used. Other data are given in (Table 1). |
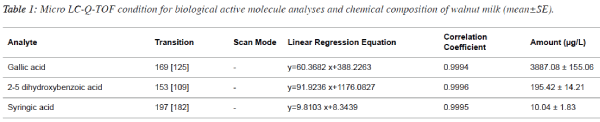 |
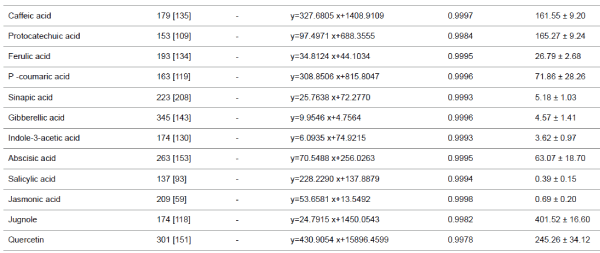 |
Cell culture and treatment conditions |
Cells were cultured in a 1:1 mixture of Dulbecco’s Modified
Eagle Medium (DMEM) and HAM’s Nutrient Mixture F12
with 5% heat-inactivated foetal bovine serum (FBS), 1% Lglutamine
and 100 IU/ml penicillin- streptomycin. Cells were
maintained at 37°C in a CO2 incubator with a humidified
atmosphere of 5% CO2 in 75 ml sterile cell culture flasks. The
experiments works began at the 5th passage of all cells. |
MTT assay |
The MTT assay was performed to determine cell viability. In
this method, DU145, MCF7, and TG/HA-VSMC cells were
sown in a 96-well sterile microplate at a density of
approximately 7500 cells/well in 200 μl of medium 24 h before
treatment. The plates were incubated in a 5% CO2 incubator at
37°C for 24 h to permit the cells to attach to the well. After
incubation, the cells were treated with 200 μl of walnut milk
and mixed medium which was filtered twice through 45
micron and 0.20 micron disposable PTFE filters (Chromex,
USA) prepared at different concentration levels of 1.25, 2.50,
5.00, 7.50, 10.0 (10 fold), 15.0, 20.0 (5-fold) and 25 % (Walnut
Milk/ Medium, V/V). After incubation for 24 and 48 h, MTT
solutions (20 μl/200 μl per well of a 5 mg/ml solution) were
added and the plates were incubated for 4 h at 37°C. The blue
formazan crystals were dissolved in DMSO (200 μl/well) and
the absorbance was measured at 490 nm with a Thermo
Multiscan Go Microplate Reader Spectrophotometer (Thermo
Scientific, USA). The calculation of absorbance for each
concentration of walnut extract mix was compared with the
water treated control. |
Tali cytometer and gene expression |
Cells were sown in 6-well sterile plates including 2 ml of
medium and routinely grown for 24 h in a CO2 incubator. After
this incubation, 5-fold- and 10-fold-diluted walnut extract
mixes (filtered through 0.45- and 0.20-micron sterile syringe
filters) diluted with medium were added to each well for 24 and 48 h. Each plate contained approximately 6 well X 5-10
105 cells. Three were used in the Tali Cytometer assay and the
others were used in gene expressions studies. |
Tali image based cytometer assays |
Cell apoptosis, viability and dead cells were determined by
Annexin V and PI (Tali® Apoptosis Kit; Life Technologies)
according to the manufacturer's instructions. After
trypsinization, the cell suspension (5-10. 105 cells) was
centrifuged at 800 g at 4°C for 2.5 min, and the supernatant
was discarded. The cell pellet was suspended in 100 μl 1X
annexin binding buffer, and 5 μl of Annexin V Alexa Fluor®
488 was added. The mixture was incubated at room
temperature in the dark for 20 min. At the end of the
incubation period, the mixture was centrifuged and the
supernatant was discarded. After suspension in 100 μl of 1X
Annexin binding buffer, an aliquot of the suspension was
mixed with 1 μl of Tali® Propidium Iodide and incubated for 5
min at room temperature. For the Tali Analysis, 25 μl of the
stained cells was loaded into a Tali® Cellular Analysis Slide
and cell viability, dead cells and apoptotic cells were
determined by Tali® Image-Based Cytometer software. |
Gene expression assays |
Isolation of total RNA and cDNA synthesis: Total RNA was
isolated from three cell lines cultured in six-well plates using
the PureLink® RNA Mini Kit (Life Technologies, USA)
according to the manufacturer's instructions. The RNA
concentrations were measured using the Qubit® Fluorometer
(Life Technologies, USA). The concentration of total RNA was
adjusted to 100 ng/μl for the synthesis of the first strand of
cDNA using a High Capacity cDNA Reverse Transcription Kit
(Life Technologies, USA). cDNA synthesis was performed
using the thermal cycler Applied Biosystems® Veriti® (Step 1:
25°C, 10 min; Step 2: 37°C, 120 min; Step 3: 85°C, 5 min).
The cDNA was stored at -20°C for subsequent steps of the
analysis procedure. |
Quantitative real-time PCR (qRT-PCR) analysis: Expression levels of antioxidant enzymes [CuZn-superoxide
dismutase (SOD), catalase (CAT), glutathione peroxidase
(GPX), Mn-SOD], apoptosis inhibitor groups [survivin, livin,
X-linked inhibitor of apoptosis (XIAP), inhibitor of apoptosis
protein (c-IAP1, c-IAP2), B-cell lymphoma 2 (BCL-2), B-cell
lymphoma-extra-large (BCL-XL)], apoptosis group [tumour
necrosis factor alpha (TNF-A), tumour suppressor (P53),
apoptosis regulator (BAX), apoptotic protease activating factor
1 (APAF-1), cytochrome C (Cyt-C), caspase 3] and cell cycle
proteins [cyclin-dependent kinase inhibitor (P21Cip1), cyclindependent
kinase inhibitor 1B (P27Kip1), cyclin-D1
(CCND1)] genes in response to walnut extract exposure were
analysed by qRT-PCR using the SYBR® select master mix
(Life Technologies, USA) on an ABI 7500 Real-Time PCR system (1 cycle of 2 min at 50°C and 10 min at 95°C followed
by 40 cycles of denaturation at 95°C for 15 s, annealing and
extension at 60°C for 1 min) with the primer pairs shown in
Table 2. Gene expression was determined as the relative fold
change compared to the control and normalized to GADPH: F
(5’-TTGGTATCGTGGAAGGACTCA-3’) and (5’-
TGTCATCATATTTGGCAGGTTT-3’) mRNA expression. The
comparative cycle threshold (Ct) method (User Bulletin 2,
Applied Biosystems, CA) was used to analyse the expression
levels of the mRNAs. In addition, differences in the degree of
the relative fold change resulting from gene expression due to
walnut extract applications were compared using analysis of
variance (ANOVA) with Duncan's separation of means test
using SPSS 18 software at a significance level of p ≤ 0.05. |
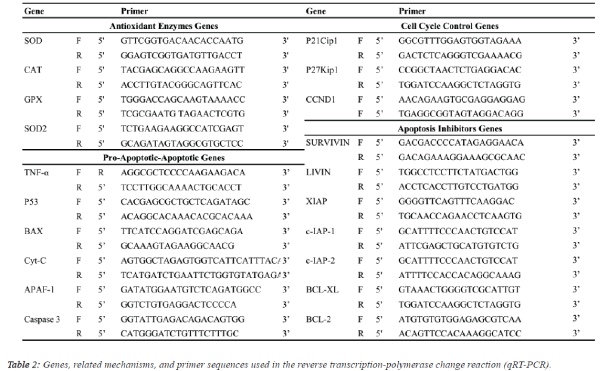 |
Results |
Phenolic compounds, plant hormones, quercetin and
juglone |
The micro LC-ESI-Q-TOF analysis of a mixture of aqueous
extracts of walnut sap, male flowers and the inner membrane
of walnut fruit revealed the presence of several phenolic acids,
plant hormones, and flavonoid derivatives. Five plant
hormones, eight phenolic acids, and quercetin (flavonoid) were
quantified. Although juglone (5 hydroxy-1,4- naphthoquinone)
has low solubility and volatile characteristics, it was present in
walnut milk extract. While ABA was the major plant hormone
(63.07 ± 18.70 μg/L), JA and SA were present at trace levels. Walnut milk exhibited a rich phenolic acid profile, in which
gallic acid was the major compound (3887.08 ± 155.06 μg/L),
followed by 2-5 dihydroxybenzoic acid (195.42 ± 14.21 μg/L).
Syringic acid was a minor compound, present at 10.04 ± 1.83
μg/L (Table 1). In this study, quercetin and juglone were the
other major components among the quantified plant
compounds, present at 245.26 ± 34.12, 401.52 ± 16.60 μg/L,
respectively. |
Determining the effective dose and time of walnut
milk |
The first step in determining the influence of a walnut extract
treatment was to calculate the effect of walnut milk (WM) on the viability and growth inhibition of cancer and normal cells.
Following treatment with increasing concentrations of walnut
milk at time points, the cells were assayed with MTT to
determine the growth inhibition and lethal potential of WM.
We observed the ability of aqueous WM to lower the viability
of cancer cells, including human breast (MCF 7) and prostate
(DU 145) adenocarcinoma cell lines. The cancer cell lines
MCF7 and DU 145 were on average inhibited to a higher
degree than the normal cell line TG/HA-VSMC. While a
significant dose- and time-dependent inhibition were observed
in both cancer cells, there was no significant correlation
between cell viability and doses of walnut extract in normal
cells at both incubation times. The cell viability in walnut
milk-treated MCF 7 and DU 145 cells showed a significant
decrease of 7.5-25% and 2.5-25% walnut milk concentrations
after 24 and 48 hours of exposure compared with their
respective control group (Figure 1). However, at 48 hours, the
inhibitory effect of walnut milk was 4.25- and 2.69-fold higher
than at 24 hours in MCF 7 and DU 145 cells, respectively. At
48 hours treatment, the IC50 and IC80 concentrations of
walnut milk were calculated as 11.34-20.39 % (y=-3.3168x +
87.63; R2=0.932) and 10.54-20.34% (y=-3.1587x+83.316;
R2=0.908) in MCF7 and DU 145 cells, respectively. The
inhibitory effects for the 10-fold (10%) and 5-fold (20%)
diluted concentrations of the walnut milk were 21.6-31.4% and
30.3-72.6 inhibition compared to controls for the MCF 7 cells;
12.7-47.4% and 46.4-64.2% inhibition for the DU 145 cells;
and 16.1-16.5% and 12.9-15.5% inhibition for the normal
TG/HA-VSMC cells (Figure 1). |
To further estimate the anticancer properties of WM, we
wanted to investigate its role in cell death mechanisms and its
selectivity to cancer cells. Our results revealed that WM
specifically induces cell death in both MCF 7 and DU 145
cancer cells in a dose- and time-dependent manner, as shown
by the increase in red fluorescence signal sources from
propidium iodide-positive cancer cells exposed to WM (Figure
2). Additionally, this effect was selective, as healthy smooth
muscle cells remained unaffected by WM treatment at the same
concentrations and time-points (Figures 1 and 2). These
outcomes were confirmed using image-based cytometry to
assess the percentage of viable, dead and apoptotic cells. In the
Tali analysis, the cells were stained with the annexin V–Alexa
Fluor® 488 conjugate for determining the apoptotic cell
population. Annexin V and propidium iodide (PI) stained cells
that were dead radiated red or yellow signals (annexin V-/PI+or
annexin V+/PI+, respectively), and irradiated green signals
when apoptotic (annexin V+/PI-). In this study, while there
were no significant changes in cell viability observed in
smooth muscle cells at all-time points after WM treatment,
compared with the controls, the live cell population of both
cancer cells was significantly reduced with increased WM
concentration under the same experimental conditions. We
determined a 15-26% and 1-27 increase in PI and annexin V-PI
positive dead cells following 48 hours of WM exposure, and a
24-47% and 47-60% annexin-V positive increase in the same
DU 145 and MCF 7 cells, confirming the induction of the
apoptosis pathway, respectively (Figure 2). |
Gene expression profiles in apoptosis signalling
induced by WM |
Several therapies used to treat cancer, such as chemotherapy, γ-
irradiation, immunotherapy and alternative medicine, have an
antitumor effect principally by activating apoptosis in cancer
cells. Several anticancer agents, especially phenolic
compound-inducible molecules, have been linked to the
activation of apoptosis signal transduction pathways in cancer
cells. For this reason, we investigated the apoptotic effect of
WM on cancer and healthy cells using gene expression profiles
of four principle components of the intrinsic pathway:
antioxidant, apoptosis inhibitor, pro-apoptotic and cell cycle. |
Oxidative stress is a key factor in the stimulation of several cell
death processes, especially apoptosis. The antioxidant genes
are rapid and sensitive biomarkers that monitor oxidative stress
in tissue and cells. Significant increases in SOD, CAT, GPX
and SOD2 gene expression were observed in DU 145 cells at
both treatment times after WM treatment (Figure 3). These
increases were observed in both reactive oxygen species
scavenger genes (cytosolic CuZn-SOD, CAT and
mitochondrial Mn SOD) in a range of approximately 1.6- to
3.7-fold, and the lipid peroxidation preventive gene (GPX) in
the range of 1.5- to 5.6-fold. |
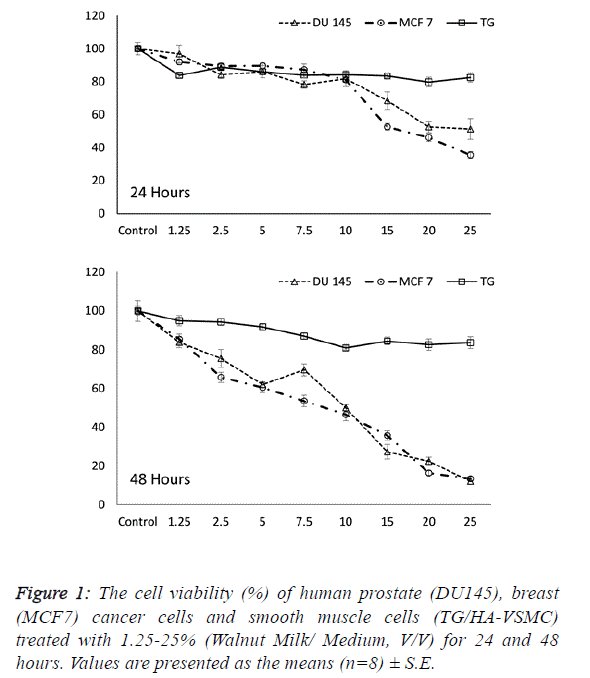 |
Our results indicate that the overexpression of antioxidant
genes could not prevent the reduction in viability (Figures 1-2),
signifying that the apoptotic effect of WM in DU 145 cells is
correlated with oxidative damage. In MCF 7 cells because no
differences were observed among control and WM treated groups in the qRT-PCR data for SOD, SOD2 and CAT genes,
the significant increases (15.5- to 23.3-fold) were determined
only in the GPX gene for the 5- and 10-fold diluted WM
treatment at 48 hours compared to the control. This indicates
that WM induced lipid peroxidation and produced hydrogen
peroxide radicals in MCF 7 cells. WM treatment did not cause
oxidative stress in normal cell lines, in contrast to cancer cell
lines. Moreover, lower expression levels of antioxidant genes
were detected in all genes, with two exceptions (Figure 3). |
To determine the apoptotic mechanisms under the effect of
WM in IC50 and IC80 doses, selected genes belonging to the
apoptosis inhibitor, apoptosis activator and cell cycle control
groups were assessed by qRT-PCR analysis. Our results
indicate that the apoptotic effect of WM was particularly
strong after 48 hours of treatment. In DU 145 cells, while
apoptosis inhibitor genes exhibited slight expression levels,
except cIAP2, apoptosis regulator genes of the intrinsic
apoptosis pathway and BAX were overexpressed (26.5-fold at
48 hours) and triggered the release of Cyt-C (10.8-fold) from
mitochondrial membranes. This gene linked the APAF 1 (2.6-
fold) and activated caspase 3 (5.1-fold). Additionally, the G1/S
transition checkpoint gene p21cip1 (overexpressed 19.5-fold)
and the cell cycle arrest p27Kip1 gene stopped the cycle in the
G1 phase. In Du 145 cells, the combined effect of these genes
completed the apoptosis cycle, especially after 48 hours of
WM treatment (Figures 4 and 5). |
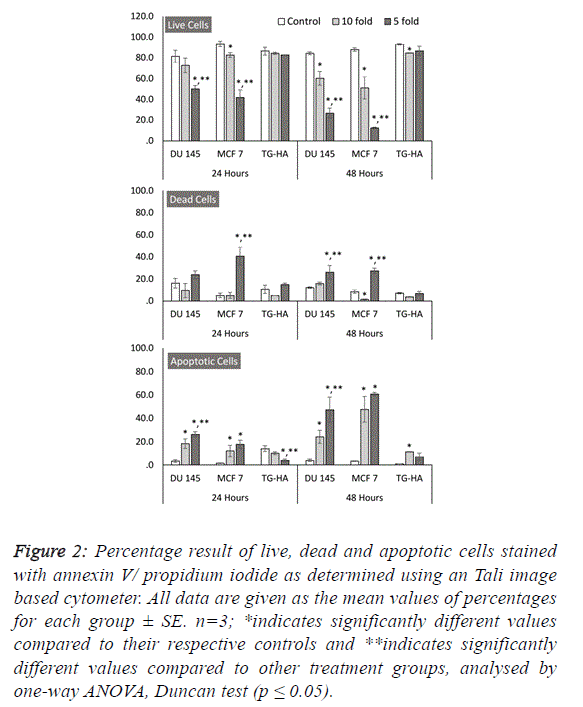 |
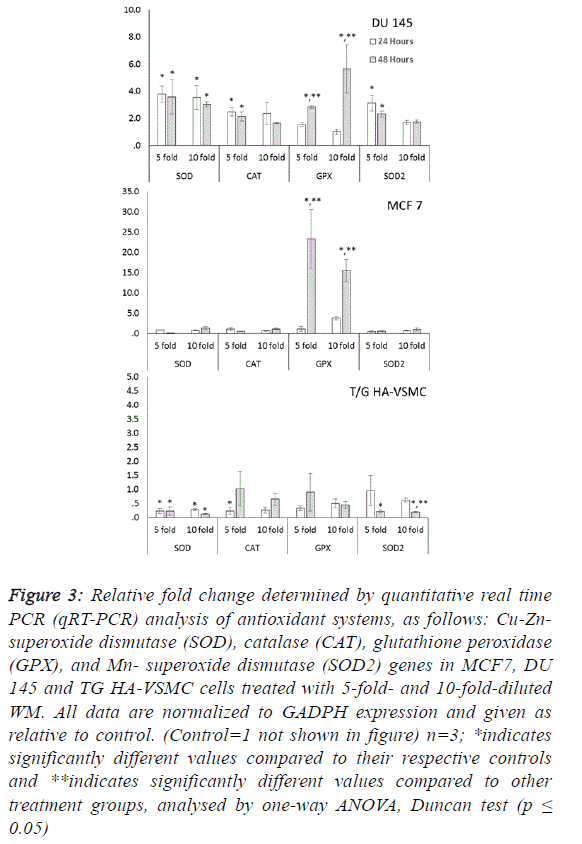 |
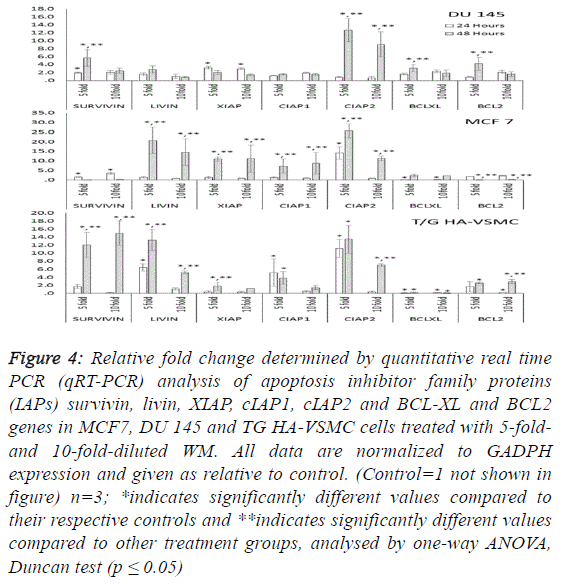 |
Treatment of MCF-7 with the 5-fold-diluted WM effectively
increased the expression of apoptosis inhibitor mRNA levels,
including the IAP groups and livin genes (Figure 4).
Conversely, in the same conditions, BCL-2 gene expression
was suppressed, and this reduction was triggered by the
increase in pro-apoptotic genes (BAX, APAF1, Cyc-C and
Caspase 3) belonging to the intrinsic apoptosis pathway
approximately in the range of 6.9- to 27.9-fold (Figure 5).
While a significant increase was also observed for tumour
suppressor P53 and tumour necrosis factor TNFα genes, the
cell cycle control genes did not exhibit dose- and timedependent
expression. In this study, normal cells were also
affected by WM treatment, and Cyc-C and APAF 1 were
induced by WM treatment. However, the BAX and Caspase 3
genes were unaffected by this increase, in addition to all
apoptosis inhibitor genes except for BCl-XL and XIAP, which
were overexpressed especially after 48 hours of WM treatment.
There were no significant changes in the expression of other
genes responsible for the cell cycle (Figure 6). For this reason,
the apoptotic effect of WM on healthy cells remained at a low
level. |
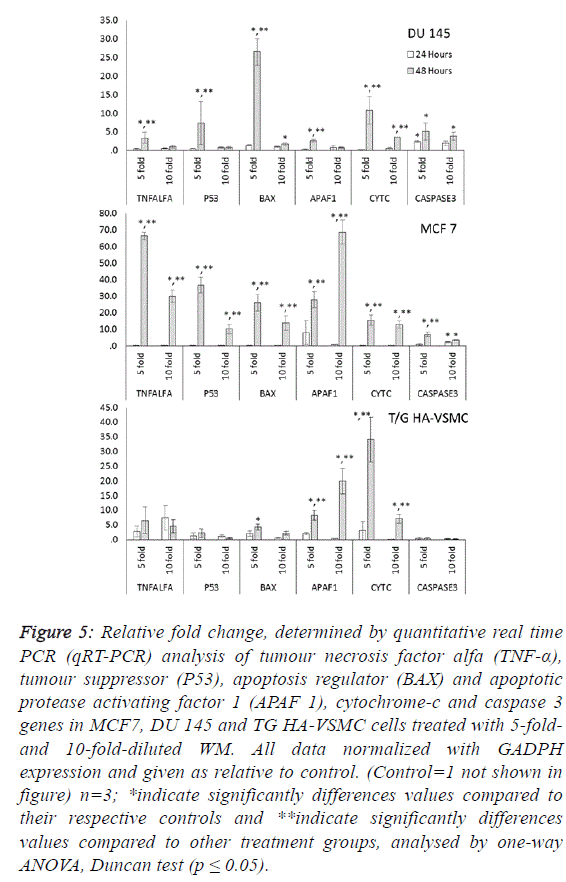 |
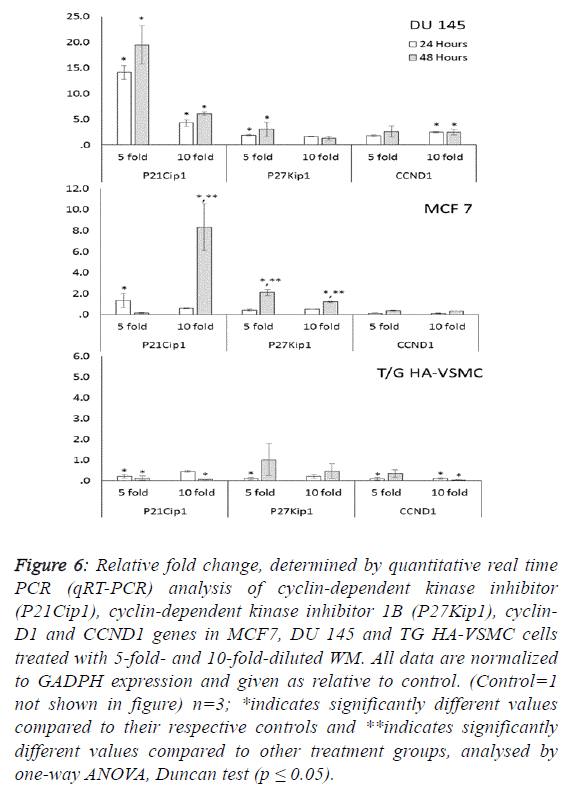 |
Discussion |
Although single and complex extracts obtained from walnut
(Juglans regia L.) seeds, green husks and leaves exert an
anticarcinogenic effect, including antiproliferative and growth
inhibition of some cancer cells [1,18-20], the underlying
mechanisms and molecular targets remain unclear. In this
report we determined for the first time the selective anticancer
capability and effect on cell signalling belonging to the
intrinsic apoptosis pathway of WM, a special walnut drink, in
human breast (MCF7) and prostate (DU145) cancer cell lines
and a non-cancerous (TG-HA-VSMC) cell line. |
The LC-Q-TOF analysis of WM revealed a rich phenolic
content compared to several plant parts (leaf, bloom, green
husk and seed) of walnut trees. Additionally, we detected four
plant hormones, especially ABA, in WM. This finding is in
agreement with several studies of the phenolic composition of
different parts of walnut trees, with the exception of the plant
hormones, in which the phenolic compounds were reported to
be catechin, epicatechin, caffeic, chlorogenic, ellagic, ferulic,
gallic, sinapic, protocatechuic, syringic and vanillic acids;
myricetin, and juglone from the walnut green husk [17],
juglone, p-coumaric, caffeic, gallic, ellagic, syringic, ferulic
and sinapic acids for walnut seeds [4,14] and nine phenolic
complexes, three hydroxycinnamic acid derivatives and six flavonols, especially heterosides of quercetin, for walnut leaves
[21]. Several cell culture and animal model studies revealed
that biologically active secondary metabolites, such as phenolic
acids and quercetin, are the main phytochemicals with
antioxidant and antiproliferative characteristics in walnut
plants [1,22,23]. Phenolic acids move easily through the cell
membrane and participate in several biochemical reactions,
such as the Fenton reaction, with their redox properties, and
play an important role in scavenging free radicals, quenching
singlet oxygen, and chelating heavy metals in antioxidant
defence mechanisms of cells [24]. It is well documented that
the polyphenols, flavonoids and plant hormones such as gallic
acid [25], ellagic acid [26], chlorogenic acid, epicatechin [7],
quercetin [27,28] and ABA [29,30] could cause apoptosis or
trigger several anticancer mechanisms in different cell lines
and animal models. |
Our results indicated that WM treatment effectively reduced
the viability of cancer cells and induced apoptosis in a doseand
time-dependent manner, while there were no significant
changes in the percentages of live, apoptotic and dead noncancerous
cells, according to the MTT and Tali Cytometer
Assays (Figures 1 and 2). Our results confirmed previous
research [1] conducted in A-498, 769-P and Caco-2 cells,
indicating that the walnut extract caused significant cell growth
inhibition in a concentration-dependent manner and has no
potent cytotoxic ability or toxic effects on healthy cells. In this
study, the two and four fold increase in the Annexin V stained
cells compared to Annexin V/PI positive and PI positive in
cancerous cell populations indicated that the marked decreases
in cell viability as determined by the MTT assay arose from
caspase-dependent apoptosis. The phosphatidylserine located
on the cytoplasmic surface of the cell membrane in living cells
is translocated from the inner to the outer membrane in
apoptotic cells via caspase activation. Annexin V stains
phosphatidylserine only in the outer membrane, and green
irradiated cells are apoptotic [31,32]. Apoptosis is a
programmed cell death, and almost all targeted and selective
anticancer therapy has been connected with stimulation of
apoptosis signalling pathways in cancer cells, such as the
intrinsic and/or extrinsic pathway [33]. Caspases are one of the
major players of apoptosis, which act as common apoptotic
molecules in a number of different substrates in the cytoplasm
in various forms of cell death [34]. |
Both the intrinsic and extrinsic apoptosis pathways are closely
related to different types of caspase activations, and these
mechanisms regulate gene expression, including antioxidant,
apoptosis inhibitor, apoptotic and cell cycle arrest genes.
Reactive oxygen species (ROS)-mediated oxidative stress is a
generalized phenomenon in most cancer therapy-induced cell
damage. The induction role of oxidative stress in different
apoptosis mechanisms has been well documented [35]. Earlier
studies have recommended that cancer cells, with different
chemical environments in the cytoplasm, are more dependent
on cellular response processes against oxidative damage and
have used this property to selectively target cancer cells [36].
While we observed a significant increase in all antioxidant
gene expression at both treatment time and dose in DU 145 cells, only GPX was overexpressed in MCF 7 cell lines under
the same treatment. Antioxidant enzymes in non-cancerous cell
lines did not significantly affect the MW application. In DU
145 cells, the increases in SOD, CAT, GPX and SOD2 gene
expression levels at both concentrations can be explained by
the ROS scavenging roles of these antioxidant enzymes.
However, oxidative stress in MCF 7 cells was more obvious
than DU 145 due to decreased SOD and CAT levels, causing
insufficient scavenging of H2O2 radicals. Our hypothesis that
overexpression of the GPX gene is the precursor of an excess
amount of hydroxyl radicals, which is closely related to lipid
peroxidation in MCF 7 cells. Increased ROS and hydroxyl
radical levels in the cells are a potent activator of lipid
peroxidation and DNA damage and high concentrations are
known to affect cellular homeostasis, which ultimately leads to
apoptotic/ necrotic cell death [37]. Additionally, ROS and
hydroxyl radicals mediate metabolic instability in the cells,
possibly triggering apoptosis by the activation of several
related genes in apoptosis pathway signalling, which is linked
to cellular damage and glutathiolation levels [38,39]. |
WM was associated with different apoptosis signalling patterns
in two cancer cell lines. In DU 145, only the survivin and cIAP
2 genes were significantly expressed by WM treatment at a
high dose, especially at 48 hours. Other apoptosis inhibitors
were slightly increased, but the apoptosis regulator BAX gene
was overexpressed and this signal triggers the release of Cyt-C
from mitochondrial membranes. Finally, a 3-fold increase in
APAF1 protein and Cyt-C complex activated Caspase 3
completed the intrinsic apoptosis circle. On the other hand, we
observed a significant increase in P21Cip1 and p27Kip1 gene
expression in DU145 cells. These cell cycle arrest genes are
closely related to P53 and BAX [40]. Biotic and abiotic cellular
stresses initiate induction of p21 expression by both p53-
dependent and -independent mechanisms [40]. The effected
mechanisms of overexpression of p21 include C6 –ceramideinduced
apoptosis in the human hepatoma (Hep3B) cell line. In
that study, overexpression of p21 triggered the proapoptotic
protein Bax, thus modulating the molecular ratio of Bcl-2/ Bax
in Hep3B cells [41]. Similarly, p21 and Bax were
overexpressed and resulted in effective apoptosis in Hep3B
cells exposed to retinoic acid [42]. In this study, we thought
that WM induced apoptosis as observed by the Tali cytometer
could be caused by crosstalk to cell cycle arrest and intrinsic
apoptosis pathway signalling in human prostate cancer (Du
145) cells. Cell cycle arrest signals play a major role in the
apoptosis pathway and the probable function of p21 that
triggers molecules responsible for regulating BAX and other
apoptotic molecules in the inhibition of cyclin-dependent
protein kinase and causes growth arrest in the G1/S sub-phase
of the cell cycle [43]. |
Human breast cancer (MCF 7) cells expressed high levels of
the inhibitor of apoptosis protein family (IAP) and proapoptotic
genes in an extended exposure (48 h) to WM
treatment. The major role of IAPs is caspase inhibition [44,45]
but they also affect several cell survival processes such as cell
division, cell cycle progression, and signal transduction
pathways [46]. They are also a good biological indicator to monitor cell damage such as lipid peroxidation, DNA damage,
and disrupting survival proteins due to significant expression,
especially against stimuli of death signalling [47]. In this study,
although the IAPs group genes were significantly
overexpressed at both high doses and long-term application,
we observed a number of death cells and apoptotic bodies by
MTT and Tali assay in a population of MCF 7 cells. For this
reason, the increases in IAPs expression levels can be
explained by the protective roles due to enhanced efficiency of
the cell survival systems of cells. However, it could be said that
the marked increases in apoptotic cells can be explained by
suppression of the BCL 2 genes by long exposure to high
concentrations of WM. In the MCF 7 cell line, our results
indicated that P53 gene expression was upregulated by WM
treatment and anti-apoptotic Bcl-2 was downregulated,
whereas the expression of pro-apoptotic protein BAX, Cyt-C,
APAF 1 and Caspase 3 were upregulated in cells exposed to
WM, which are considered as excellent signals to determine
the intrinsic apoptosis pathway [48,49]. We observed that WM
treatment did not cause oxidative stress in TG/HA-VSMC cell
lines at both treatment dose and time. While significant
increases were determined, the expression levels of IAPs group
genes and anti-apoptotic BCL2 as well as pro-apoptotic
APAF1 and Cyt-C, the main player of apoptotic genes, such as
Caspase 3 and P21, were significantly down regulated. We
thought that in TG/HA-VSMC cells, overexpressed IAPs genes
allow enough time to repair the survival molecules, in the
meantime increasing the level of BCL2 and suppressing
caspase 3 activation signals that occur as a response to WM
application, thus preventing apoptosis and non-cancerous cells
remaining viable. |
A number of studies conducted on both cell lines and animal
tissues emphasised that the natural plant extract, with multiple
bioactive molecules, has several advantages compared to single
therapeutics [1,50]. Natural aqueous extracts are suitable for
human consumption and most of them may be administered
orally. Moreover, rich bioactive molecules within natural
extracts not only effect many survival mechanisms in cells via
a synergistic enhancement of anticancer activities but also may
possibly decrease the resistance to chemotherapy [51]. In
conclusion, our results demonstrate that 5-fold-diluted WM,
especially at 48 hours, is selective in inducing apoptotic cell
death in both human breast (MCF7) and prostate (Du 145)
cancer cells by targeting intrinsic apoptosis signalling
pathways. The same experimental exposure is well tolerated by
non-cancerous TG/HA-VSMC cells. Compared to previous
findings from studies on the anticancer effect of phenolic
compounds on cancer cells, the mechanisms of action for WM
seem more likely related to its phenolic ingredients. According
to our findings, we suggest that WM is a potential anticancer
agent with a selective apoptotic potential and special bioactive
chemical constituents against at least human prostate and
breast cancer. |
Acknowledgement |
The authors are grateful to Prof. Dr. Yener YORUK and the
Technology Research and Application Centre (TUTAGEM),
which is funded by the T.R. State Planning Organization
(Project Number: 2011K120390), for providing the laboratory
equipment. We acknowledge Ucan Adam (Denizli, Turkey) for
supplying us with walnut milk. |
References |
- Carvalho M, Ferreira PJ, Mendes VS, Silva R, Pereira J, et al. Human cancer cell antiproliferative and antioxidant activities of Juglansregia L. Food ChemToxicol 2010; 48: 441-447.
- Cai Q, Rahn RO, Zhang R. Dietary flavonoids, quercetin, luteolin and genistein, reduce oxidative DNA damage and lipid peroxidation and quench free radicals. Cancer letters 1997; 119: 99-107.
- Fiuza SM, Gomes C, Teixeira LJ, Da Cruz MG, Cordeiro MNDS, et al. Phenolic acid derivatives with potential anticancer properties––a structure–activity relationship study. Part 1: Methyl, propyl and octyl esters of caffeic and gallic acids. Bioorg Med Chem 2004; 12: 3581-3589.
- Colaric M, Veberic R, Solar A, Hudina M, Stampar F. Phenolic acids, syringaldehyde, and juglone in fruits of different cultivars of Juglansregia L. J Agr Food Chem 2005; 53: 6390-6396.
- Oliveira I, Sousa A, Ferreira IC, Bento A, Estevinho L, et al. Total phenols, antioxidant potential and antimicrobial activity of walnut (Juglansregia L.) green husks. Food ChemToxicol 2008; 46: 2326-2331.
- Liu RH. Health benefits of fruits and vegetables are from additive and synergistic combination of phytochemicals. Am J ClinNutr 2003; 78: 517-520.
- Ramos S, Alía M, Bravo L, Goya L. Comparative effects of food-derived polyphenols on the viability and apoptosis of a human hepatoma cell line (HepG2). J Agr Food Chem2005; 53: 1271-1280.
- BlomhoffR, Carlsen MH, Andersen LF, Jacobs DR. Health benefits of nuts: potential role of antioxidants. Brit J Nutr 2006; 96: 52-60.
- Pereira JA, Oliveira I, Sousa A, Valentão P, Andrade PB, et al. Walnut (Juglansregia L.) leaves: phenolic compounds, antimicrobial activity and antioxidant potential of different cultivars. Food ChemToxicol 2007; 45: 2287-2295.
- Zhanga Z, Liaoc L, Moored J, Wua T, Wanga Z. Antioxidant phenolic compounds from walnut kernels. Food Chem 2009; 113: 160-165.
- Pulido R, Bravo L, Saura-Calixto F. Antioxidant activity of dietary polyphenols as determined by a modified ferric reducing/antioxidant power assay. J Agr Food Chem 2000; 48: 3396-3402.
- Silva BM, Andrade PB, Valentão P, Ferreres F, Seabra RM, et al. Quince (Cydoniaoblonga Miller) seed (pulp, peel, and seed) and jam: antioxidant activity. J Agr Food Chem 2004; 52: 4405-4712.
- Sroka Z, Cisowski W. Hydrogen peroxide scavenging, antioxidant and anti-radical activity of some phenolic acids. Food ChemToxicol 2003; 41: 753-758.
- Fukuda T, Ito H, Yoshida T. Effects of the walnut polyphenol fraction on oxidative stress in type 2 diabetes mice. Bio Factors 2004; 21: 251-253.
- Li L, Tsao R, Yang R, Liu C, Zhu H, et al. Polyphenolic profiles and antioxidant activities of heartnut (Juglansailanthifoliavar.cordiformis) and Persian walnut (Juglansregia L.). J Agr Food Chem 2006; 54: 8033-8040.
- Espín JC, Soler-Rivas C, Wichers HJ. Characterization of the total free radical scavenger capacity of vegetable oils and oil fractions using 2,2-diphenyl-1-picrylhydrazyl radical. J Agr Food Chem 2000; 48: 648-656.
- Stampar F, Solar A, Hudina M, Veberic R, Colaric M. Traditional walnut liqueur – cocktail of phenolics. Food Chem 2006;95: 627-631.
- Kaur K, Michael H, Arora S, Härkönen PL, Kumar S. Studies on correlation of antimutagenic and antiproliferative activities of Juglansregia L. J Environ PatholToxicolOncol 2003; 22: 59-67.
- Hardman WE, Ion G. Suppression of implanted MDA–MB 231 human breast cancer growth in nude mice by dietary walnut. Nutr Cancer 2008; 60: 666-674.
- Yang J, Liu R, Halim L. Antioxidant and antiproliferative activities of common edible nut seeds. Food SciTechnol 2009; 42: 1-8.
- Amaral J, Seabra RM, Andrade PB, Valentão P, Pereira JA, et al. Phenolic profile in the quality control of walnut (Juglansregia L.) leaves. Food Chem 2004; 88: 373-379.
- Almeida IF, Fernandes E, Lima JL, Costa PC, Bahia MF. Walnut (Juglansregia) leaf extracts are strong scavengers of pro-oxidant reactive species. Food Chemistry 2008; 106: 1014-1020.
- Labuckas DO, Maestri DM, Perelló M, Martínez ML, Lamarque AL. Phenolics from walnut (Juglansregia L.) kernels: Antioxidant activity and interactions with proteins. Food Chem 2008; 107: 607-612.
- Costa RM, Magalhães AS, Pereira JA, Andrade PB, Valentão P, et al. Evaluation of free radical scavenging and antihemolytic activities of Cydoniaoblonga leaf. A comparative study with green tea (Camellia sinensis). Food ChemToxicol 2009; 47: 860-865.
- Yáñez J, Vicente V, Alcaraz M, Catillo J, Benavente-García O, et al. Cytotoxicity and antiproliferative activities of several phenolic compounds against three melanocytes cell lines: relationship between structure and activity. Nutr Cancer 2004; 49: 191-199
- Han DH, Lee MJ, Kim JH. Antioxidant and apoptosis-inducing activities of ellagic acid. Anticancer Res 2006; 26: 3601-3606.
- Mertens-Talcott S, Talcott ST, Percival SS. Low concentrations of quercetin and ellagic acid synergistically influence proliferation, cytotoxicity and apoptosis in MOLT-4 human leukemia cells. J. Nutr. 2003; 133: 2669-2674.
- Nguyen TTT, Tran E, Nguyen TH, Do PT, Huynh TH, et al. The role of activated MEK-ERK pathway inquercetin-induced growth inhibition and apoptosis in A549 lung cancer cells. Carcinogenesis 2004; 25: 647-665.
- Li HH, Hao RL, Wu SS, Guo PC, Chen CJ, et al. Occurrence, function and potential medicinal applications of the phytohormoneabscisic acid in animals and humans. Biochemical Pharmacol 2011; 82: 701-712.
- Vildanova MS, Savitskaya MA, Onishchenko GE, Smirnova EA. The effect of plant hormones on the components of the secretory pathway in human normal and tumor cells. Cell Tissue Biol 2014; 8: 407-415.
- Luchetti F, Canonico B, Curci R, Battistelli M, Mannello F, et al., Melatonin prevents apoptosis induced by UV-B treatment in U937 cell line. J Pineal Res 2006; 40: 158-167.
- Salucci S, Burattini S, Battistelli M, Baldassarri V, Curzi D, et al. Melatonin prevents chemical-induced haemopoietic cell death. Int. J MolSci 2014; 15: 6625-6640.
- Fulda S, Debatin KM. Extrinsic versus intrinsic apoptosis pathways in anticancer chemotherapy. Oncogene 2006; 25: 4798-4811.
- Degterev A, Boyce M, Yuan J. A decade of caspases. Oncogene 2003; 22: 8543-8567.
- Simon HU, Haj-Yehia A, Levi-Schaffer F. Role of reactive oxygen species (ROS) in apoptosis induction. Apoptosis2000; 5: 415-8
- Raj L, Ide T, Gurkar AU, Foley M, Schenone M, et al. Selective killing of cancer cells by a small molecule targeting the stress response to ROS. Nature 2012; 481: 534-534.
- Winyard PG, Moody CJ, Jacob C. Oxidative activation of antioxidant defence. Trends BiochemSci 2005; 30: 453-461.
- Kim H, Kim YN, Kim H, Kim CW. Oxidative stress attenuates Fas-mediated apoptosis in Jurkat T cell line through Bfl-1 induction. Oncogene 2005; 24: 1252-1261.
- Srivastava RK, Rahman Q, Kashyap MP, Singh AK, Jain G, et al. Nano-titanium dioxide induces genotoxicity and apoptosis in human lung cancer cell line, A549. Hum ExpToxicol 2013; 32:153-166.
- Gartel AL, Tyner AL. The role of the cyclin-dependent kinase inhibitor p21 in apoptosis. Mol Cancer Ther 2002; 1: 639-649.
- Kang KH, Kim WH, Choi KH. p21 promotes ceramide induced apoptosis and antagonizes the antideath effect of Bcl-2 in human hepatocarcinoma cells. Exp. Cell Res 1999; 253: 403-412.
- Hsu SL, Chen MC, Chou YH, Hwang GY, Yin SC. Induction of p21(CIP1/ Waf1) and activation of p34(cdc2) involved in retinoic acid-induced apoptosis in human hepatoma Hep3B cells. Exp Cell Res 1999; 248: 87-96.
- Satyanarayana A, Hilton MB, Kaldis P. p21 Inhibits Cdk1 in the absence of Cdk2 to maintain the G1/S phase DNA damage checkpoint. MolBiol Cell 2008; 19: 65-77.
- Nunez G, Benedict MA, Hu Y, Inohara N. Caspases: the proteases of the apoptotic pathway. Oncogene 1998: 17: 3237-3245.
- Deveraux QL, Stennicke HR, Salvesen GS, Reed JC. Endogenous inhibitors of caspases. J ClinImmunol 1999; 19: 388-398.
- La Casse EC, Baird S, Korneluk RG, MacKenzie AE. The inhibitors of apoptosis (IAPs) and their emerging role in cancer. Oncogene 1998; 17: 3247-3259.
- Schimmer AD, Dalili S. Targeting the IAP family of caspase inhibitors as an Emerging Therapeutic Strategy. Hematology Am. Soc. Hematol. Educ. Program. 2005; 215-219.
- Chau BN, Cheng EH, Kerr DA, Hardwick JM. Aven, a novel inhibitor of caspase activation, binds BclxL and Apaf-1. Mol Cell 2000; 6: 31-40.
- Cory S, Adams JM. The Bcl2 family: regulators of the cellular life-or-death switch. Nat Rev Cancer 2002; 2: 647-656.
- Ovadje P, Ma D, Tremblay P, Roma A, Steckle M, et al. Evaluation of the efficacy & biochemical mechanism of cell death induction by Piper longum extract selectively in in-vitro and in-vivo models of human cancer cells. PLoS ONE. 2014; 9(11): e113250.
- Foster BC, Arnason JT, Briggs CJ. Natural health products and drug disposition. Annu Rev PharmacolToxicol 2005; 45: 203-226.
|